The Denver Convergence-Vorticity Zone -
From A Storm Chaser’s Perspective
| |||||||||||||||||||||||||||||||||||||||||||||||||||||||||||||||||||||||||||||||||||||||||||||||||||||||||||||||||||||||||||||||||||||||||||||||||||||||||||||||||||||||||||||||||||||||||||||||||||||||||||||||||||||||||||||||||||||||||||||||||||||||||||||||||||||||||||||||||||||||||||||||||||||||||||||||||||||||||||||||||||||||||||||||||||||||||||||||||||||||||||||||||||||||||||||||||||||||||||||||||||||||||||||||||||||||||||||||||||||||||||||||||||||||||||||||||||||||||||||||||||||||||||||||||||||||||||||||||||||||||||||||||||||||||||||||||||||||||||||||||||||||||||||||||||||||||||||||||||||||||||||||||||||||||||||||||||||||||||||||||||||||||
STEVE HAMILTON
Preface
The Denver Convergence-Vorticity Zone has been studied since 1981 by
various meteorologists, storm chasers and amateur weather enthusiasts.
The numerous studies and analyses of each discipline have stemmed from a
few different motivations. Meteorologists, especially those employed by
NOAA, are most interested in protecting life and property in and near
the Denver suburbs. Storm chasers wish to take advantage of film and
photographic opportunities, and share their “catch” with the public.
Amateur weather enthusiasts primarily focus on the basics of the
phenomena with a general interest in spotting and predicting
non-supercell tornadoes and severe weather events. The most
comprehensive official study of the Denver Convergence-Vorticity Zone
was done by Meteorologist Edward J. Szoke of the NOAA Earth Systems
research Laboratory in Boulder, Colorado. Mr. Szoke has compiled
extensive documentation of the wind flow patterns, overlain on terrain
maps, to support the general accepted theory of this important
warm-season circulation. An additional study was completed on the 25th
anniversary of the discovery of the Denver Convergence Vorticity Zone in
2006 by Mr. Szoke, along with Dave Barjenbruch, Robert Glancy and Robert
Kleyla of the NOAA WSFO in Boulder, Colorado.
This paper will address the Denver Convergence-Vorticity Zone,
(henceforth referred to as the “DCVZ”), from the perspective of storm
chasers. The focus will be on the nomenclature, definition and theories
of formation, and related severe weather. Finally, some strategies will
be put forth for storm chasers to consider when attempting to study and
photograph resultant non-supercell tornadoes and other circulations
which can be a focal-point for the development of larger storms farther
out on the Colorado plains.
Discussion of Nomenclature and Terminology
The DCVZ has recently been otherwise referred to as the “Denver
Cyclone”. It’s my personal preference to refer to the phenomena as a
zone of vorticity and convergence. The use of the term “cyclone” implies
that the DCVZ is formed in a similar fashion as a mid-latitude cyclone.
There are several key differences in the formation of the DCVZ compared
to a mid-latitude cyclone, although the main circulations of the two are
both cyclonic. A few of the basic differences are noted below.
First, cyclones which form in the mid-latitudes of North America are
initially formed by large-scale temperature differences, which generate
pressure gradients and result in specific wind flow patterns, advection,
convergence and synoptic scale dynamic lifting. This is known as a
baroclinic environment, (fronts, and air masses of differing temperature
and moisture are present). A cyclone is a counter-clockwise, (northern
hemisphere), circulation in association with baroclinic differentials,
(temperature, moisture). The DCVZ is a zone of converging air resulting
from wind shear as it relates to the convergence portion of the matrix.
The DCVZ is formed when low-level wind flow interacts with terrain
features in eastern Colorado. Often, the temperature field in eastern
Colorado on a DCVZ day contains no synoptic-scale differentials,
(baroclinicity), and the moisture field is consistent throughout, or
shows only minor variation, with lower dewpoints usually the result of
higher terrain rather than air mass characteristics. The key difference
lies in the fact that a baroclinic environment causes the formation of a
typical mid-latitude cyclone, while a baroclinically-induced wind field
assists in the formation of the DCVZ. In the case of the former, it is
the baroclinicity itself that forms the cyclone, while in the case of
the latter, the baroclinic environment is usually already formed when
the DCVZ is initiated. So, a DCVZ could be formed as an after-product of
a passing mid-latitude cyclone or a cold front. However, it should be
noted that a baroclinic environment is not always necessary to form a
DCVZ because it is the result of the PBL wind field interacting with
terrain, not temperature discontinuity. DCVZ can indeed form as the
result of a mid-latitude cyclone passing through the area with its
counter-clockwise circulation. This situation is more accurately known
as a Denver Cyclone.
Second, in the case of mid-latitude cyclones, the wind field is formed
due to pressure gradients, whereas the wind field in the DCVZ develops
as a result of the overall synoptic-scale pattern or possibly due to
diurnal wind flow. The difference being that the wind field is not
necessarily generated by differences in temperature or moisture across a
boundary.
Terms used to describe the formation of the DCVZ include:
Mesoscale – a meteorological scale referring to events and air masses
smaller than synoptic scale. In the case of the DCVZ, mesoscale refers
to the convergence boundary associated with the event, (20 to 200 km)
and to the thunderstorms which sometimes form on the boundary, (20 to 30
km).
Convergence – a meeting of winds from opposing directions, sometimes of
different intensities. The angle at which the winds meet in the DCVZ can
help determine the intensity of the uplift and whether the resultant
updrafts will be successful in breaking any capping inversion.
Convergence is one of the primary ways of achieving dynamic uplift,
which is critical for condensation and precipitation.
Vorticity – A spin or circulation of air present in the meso or synoptic
scales. As it relates to the DCVZ, the overall circulation is
counter-clockwise, generating “positive” vorticity. The mesoscale
circulations, or eddies, are on the order of 5 to 20 km.
Non-supercell Tornado – A violently rotating column of air that occurs
with a parent cumulus cloud in the growth stage, with vorticity that
originates in the boundary layer. The parent cloud does not contain a
preexisting mid-level mesocyclone.
Growth Stage – This refers to a cumulus cloud that is in the stage prior
to the “mature” stage. The growth stage is marked by rapid vertical
development. In the case of the DCVZ, the development is caused by
dynamic lifting of air above the area experiencing convergence.
PBL (Planetary Boundary Layer) – The layer of the atmosphere extending
from the surface to approximately 3 km above the surface. In relation to
the DCVZ, the PBL wind field is the prerequisite for the formation of
the circulation.
Landspout – A non-supercell tornado.
To conclude, a proper name for this phenomenon should be the Denver
Convergence-Vorticity Zone, versus the Denver Cyclone, referring to the
formation prerequisites of a mid-latitude cyclone. The other terms used
in descriptions of the DCVZ retain their proper meanings.
Definition and Formation
The DCVZ, as defined by the American Meteorological Society, is a
mesoscale flow feature of convergent winds, 50 to 100 km in length,
usually oriented north to south, just east of the Denver, Colorado area.
This is a general definition of the phenomenon, and its position, length
and intensity can vary greatly depending on the synoptic conditions,
temperature and moisture fields, interference from non-related synoptic
features, and prevailing wind vectors.
The prevailing theory of the formation of the DCVZ is that a south or
southeasterly wind flow interacts with the varied terrain in eastern
Colorado. One of the prominent features is an outcrop called the Palmer
Divide. This is a region of relatively high elevation, (approximately
7,800 feet) which protrudes in an east-west fashion, somewhat
perpendicular to the north-south line formed by the Rocky Mountains to
the west. The Denver metropolitan area sits in somewhat of a “bowl” of
lower elevation, to the north and west of this location. Denver is lower
than the foothills to the west, the slightly elevated terrain to the
northeast and the Palmer Divide to the south. All of these features
contribute to the formation of the DCVZ. The Denver valley serves to
enhance the deflected wind flow and concentrate it in a relatively small
area.
The formation of a DCVZ requires a strong low-level wind flow from the
south or most favorably the southeast. The geographical area involved in
the formation of a DCVZ extends from Cheyenne, WY in the north to
Colorado Springs, CO in the south. The western boundary is the foothills
of the Colorado Front Range to the west, and a line extending from Akron
to Limon, CO in the east. The Palmer Divide or ridge is located in an
east-west orientation north of Colorado Springs and south of a line from
Castle Rock to Elizabeth to Kiowa, CO.
The DCVZ is thought to form as PBL air, moving from south-southeast to
north-northwest, flows over the Palmer Divide in a general southeasterly
flow at the synoptic scale. The higher terrain serves to bend the wind
flow toward the west due to friction as it passes over the Palmer Ridge.
A statically-stable lower tropospheric condition serves to concentrate
the circulation in the PBL. The vorticity piece of the DCVZ forms as the
air is deflected due to friction over the Palmer and bent toward the
northwest. From here, some of the air can be deflected further westward
along the Cheyenne ridge to the north in the case of a large-scale DCVZ.
Otherwise, air flowing over the mountains from west to east moves down
the east slopes and meets the southeasterly flow somewhere east to
southeast of Denver. This denotes the convergence piece of the DCVZ.
The resulting severe weather forms along the convergence boundary where
dynamic uplift is most enhanced. There can also be a vast difference in
the moisture field of the mountain air and the air moving in from the
southeast, which is usually more moist.
Nearly three decades of tornado reports have been gathered in eastern
Colorado. While the levels of occurrence were down in the 1990s and
2000s, the distribution of tornadic events remained fairly uniform.
According to NOAA, greater numbers of tornadoes have occurred in the
non-mountainous counties just east of the Denver area since 1980.
Coincidentally, the DCVZ frequently forms over these counties. Weld
County has reported the highest number of tornadoes, partially because
of its larger size. Second in ranking is Adams County, followed by
Arapahoe, then Douglas. Incidentally, the NOAA data have shown that a
substantial drop in the number of tornadoes in all of these counties has
occurred in the current decade, coinciding with fewer DCVZ events.
The reasons for a southeasterly surface flow forming a DCVZ are usually
synoptic in nature. It is common for a DCVZ to form one to three days
after the passage of a Canadian cold front. These situations are more
frequent in the months of May and June, before the polar front moves too
far north. A few days after the front passes, the return flow around the
following high pressure ridge is normally from the southeast. According
to a study done by researcher and veteran storm chaser Albert Pietrycha,
a high degree of static stability is required for a cyclonic circulation
to form. That is, sinking air under the high pressure ridge. With static
stability present, the intensity of the southeasterly air flow over the
Palmer Ridge can be kept in the PBL and the full momentum can be used in
the formation of the DCVZ.
Related Severe Weather
The most intense and dangerous weather resulting from a DCVZ is the
non-supercell tornado. However, the conditions on any given DCVZ day may
or may not generate NSC tornadoes. On some DCVZ days, the formation of
the circulation and consequent convergence generates nothing more than a
few cumulus clouds, or an invisible convergence boundary where the
prevailing low-level southeast flow meets the north or northwest flow
moving south along the foothills. Referring to the 1981 Szoke study, it
has been found that on days where an ambient south or southeast surface
wind flow was present, a DCVZ formed about 80% of the time. A strong
correlation with non-supercell tornadoes was also identified. In the
month of June, Szoke found that 40% of the DCVZ days had a tornado. June
seems to be the most dominant month for the formation of a DCVZ, and
tornadoes that formed in association with a DCVZ were not associated
with any other type of severe weather. In other words, tornadoes have
been forming in the vicinity of a DCVZ under conditions which formed
ordinary, or non-mesocyclone, thunderstorms. In the Szoke study,
conclusions were drawn that June days with a DCVZ present had a 30%
chance of being “tornado days”, with the chance rising to about 60% in
the presence of a strong DCVZ.
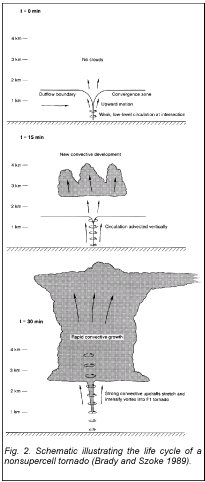
The figure above is a model of the formation of a non-supercell
tornado, developed from a Doppler radar study of the DCVZ conducted by
Szoke and Bradley in 1989. The importance of a boundary as a point of
initiation was revealed in this, and subsequent, studies. It has been
widely documented that non-supercell tornadoes can form in association
with growth-stage cumulus clouds. These rapidly-developing cumulus
clouds form along a convergence boundary such as the DCVZ, or an
interaction between low-level wind flow and an outflow boundary. As
illustrated at left, the convergence zone formation is the first phase
in the development of clouds in the region. The elements of heat,
moisture and convergence work together to form a circulation around a
vertical axis. In approximately 15 minutes, the visible cumulus cloud
forms in the rising vertical column of air above the vort point, and the
vorticity is vertically advected and imposed on the rising air, (center
frame). As the cloud develops pas the 30 minute mark, while still in
growth stage, the vorticity is stretched as the air column rises
rapidly. Air is pulled in at the base of the circulation to replace the
rising air, enhancing development. In this manner, a non-supercell
tornado forms, essentially from the ground, up. Or, more accurately,
involving the entire column of air as it rises from the ground to the
LCL and beyond.
In addition, the position of the strongest convergence can have a
profound effect on what type of severe weather develops, and its
morphology. Convergence zones that form farther west, usually in more
dry air, may only generate a few clouds as visible clues. When the zone
forms farther east, in more abundant moisture and with more intense
heating, stronger updrafts can be the result. Generally, storms forming
on the DCVZ are slow-moving compared to those forming due to other
situations. Most DCVZ storms sustain themselves in the same general
area, rather than moving off onto the plains. When these storms do
eventually get caught up in the mid-level flow, they sometimes lose
intensity after moving off the convergence zone, because they no longer
have a source of uplift. Unless such a storm can sustain its updraft in
a favorable environment, it may dissipate. A favorable environment would
be a region that has been experiencing strong diurnal heating and has
had good moisture advected into it. Another key ingredient is shear.
Unfortunately, most DCVZ days lack vertical shear due to the nature of
the preexisting conditions that formed the DCVZ in the first place. A
DCVZ will usually form on days of limited shear, especially in the PBL.
The only shear that is generated is due to the deflected wind flow,
rather than baroclinic conditions. All of the shear is at the
lower-levels of the atmosphere, in the PBL. Mid and upper-level winds
are usually weak on DCVZ days.
DCVZ Chase Strategies
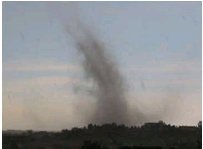
Non-supercell tornadoes can be fascinating to chase and photograph. The
main advantage in viewing/chasing these tornadoes over mesocyclonic
tornadoes is their slow movement. Supercell tornadoes can move as fast
as 50 or 60 mph. Since DCVZ tornadoes form along a convergence boundary
which sustains their growth and development, they tend to move at less
than 25 mph and sometimes appear nearly stationary.
In anticipating a DCVZ chase day, the synoptic and mesoscale features to
look for include a stable southeasterly flow at the surface, strong
diurnal heating, a capping inversion and low values of mid-level
horizontal and vertical shear.
Following frontal passage and the formation of a DCVZ, Al Pietrycha
recommends an analysis of the persistence of the gyre. It should persist
for at least 6 hours to be considered a true DCVZ. According to his
research, the longest recorded DCVZ circulation was of a duration of
about 36 hours. Surface data, satellite imagery and radar data are the
three critical elements that should be utilized when in “chase mode” on
a non-supercell tornado event along the DCVZ. According to Mr. Pietrycha
and other veteran storm chasers, the key strategy in intercepting
non-supercell DCVZ tornadoes is to locate on the DCVZ prior to the
development of rapidly growing cumulus towers. Frequently, the time from
initial cloud formation to tornadogenesis is less than 30 minutes.
Surface data are essential for determining the location of the
convergence boundary. Since temperature and humidity differences may be
minimal, use the surface wind observations to determine the strongest
area of convergence. Locating the boundary itself and the areas of
strongest convergence can be difficult using only surface observations.
This is due to the fact that there are very few “official” observation
stations east of Denver, and they are widely scattered. Also, surface
observations are only issued once per hour and mesoscale conditions can
change much more rapidly than that. It is therefore necessary to
supplement surface data with WSR-88D data. The National Weather Service
office in Boulder, CO maintains a Doppler radar facility northeast of
Denver, in perfect position for analysis of the DCVZ. As strong shear
develops in the convergence zone, this will be evident in the colors
displayed on the WSR-88D data. Storm-relative motion mode will reveal
inbound winds as green and outbound winds as red. This will show the
actual boundary, and eddies forming along it. These eddies can be a
focal point for NSC tornadoes if a cumulus tower forms over them.
High-resolution satellite data are also useful in locating the boundary
and any cumulus clouds forming on it. The high-res images are updated
every 15 minutes and will show towers going up along the convergence
boundary. It should be noted that many DCVZ events generate
non-supercell tornadoes in the early afternoon hours, between noon and 2
p.m. Therefore, shadows on the satellite images will be at a minimum.
Careful analysis of satellite data on a large non-glossy display in
low-glare conditions is usually necessary to pick up small cumulus clouds.
Non-supercell tornadoes must be viewed from the west, looking east. The
primary reason for this is their visual characteristics. Non-supercell
tornadoes rarely reach above EF-1 intensity. Thus, they do not pull as
much debris and dust from the surface up into their funnels.
Additionally, there is no condensation funnel to speak of. This gives
the tornadoes a semi-transparent appearance. If they are viewed looking
west into brighter skies, they will be nearly invisible. However,
looking east from behind the developing towers, the sun will not be an
interference, and the dark color of the dust and dirt being sucked off
the ground will contrast nicely with either blue sky or white clouds in
the background.
Forecasting tornadoes on the DCVZ is a continued challenge. There are no
definite parameters on which to base forecasts, and no obvious patterns
to the formation of storms along the boundary which produce
non-supercell tornadoes.
Conclusions
With populations growing rapidly in the Denver area, continued research
is necessary to better predict non-supercell tornadoes along the DCVZ.
Non-supercell tornadoes can reach EF-1 intensity, causing substantial
damage to homes and businesses. The rapid development of DCVZ tornadoes
is a serious challenge to their prediction. The goal of NOAA is to be
able to more accurately predict DCVZ tornadoes, while limiting the
number of false alarms, which damage credibility and undermine the
confidence of the public. I’ll propose a plan here that may aid in the
research of conditions leading to non-supercell tornadoes on the DCVZ.
With the development of GPS and mobile internet technology, it is now
possible to coordinate a team of storm chasers positioned around
developing cumulus towers on DCVZ days. My proposal would be to organize
a volunteer group of chasers based in the Denver area who would be
available to deploy on favorable days. In the chase vehicles would be a
laptop computer with mobile internet. Interactive software can be
developed to that the positions of the chase vehicles can be constantly
monitored by a coordinator at NOAA in Boulder. The GR Level 3 radar
program would be installed on each laptop in the chase vehicles. Also,
NOAA could develop a standardized instrument package to measure wind
speed and direction, temperature and humidity on each chase vehicle.
This information would also be transmitted via mobile internet back to
NOAA. In this way, a mobile mesonet of chasers could be positioned along
the DCVZ to gather data, and then document any non-supercell tornadoes
with video and digital photography. The key to accurately researching
mesoscale events such as the DCVZ is to have a network of perhaps 50
mobile observation platforms, capable of delivering a large data sample
for analysis. From this data, it may be possible to pinpoint enough
parameters on which to base a tornado forecast on DCVZ days. The
cost-benefit relationship is increasingly justifiable with the increases
in population in areas frequently affected by the DCVZ.
References
Observations Of The DCVZ Using Mobile Mesonet Data. Albert E. Pietrycha
and Erik N. Rasmussen. National Severe Storms Laboratory and Texas Tech
University. Cooperative Institute for Mesoscale Meteorological Studies,
NSSL and University of Oklahoma.
The Denver Cyclone And Tornadoes 25 Years Later: The Continued Challenge
Of Predicting Non-Supercell Tornadoes. Edward J. Szoke, NOAA Earth
System Research Laboratory/Global Systems Division, Boulder Colorado in
collaboration with the Cooperative Institute for Research in the
Atmosphere (CIRA). Dave Barjenbruch, Robert Glancy and Robert Kleyla,
NOAA National Weather Service Forecast Office, Boulder, Colorado.
|
|
|